The biosynthetic pathways of galloylglucoses and gallotannins are well-known due to the work by Georg G. Gross and collaborators in the 1990’s
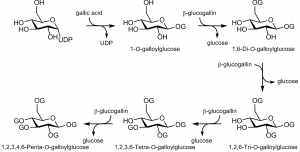
The main pathway in the formation of 1,2,3,4,6-penta-O-galloylglucose. The letter G is used to describe a galloyl unit.
The galloyl glucose and GT pathways look relatively simple, since larger tannins are synthesized by adding new galloyl groups into the smaller ones. However, there is clear position-specificity in the galloylation steps and this primarily dictates the main galloylglucose structures formed in plant cells. This knowledge is useful to take into account in the structure elucidation of the galloylglucose series.
The biogenesis of GGs (the main pathway shown in Fig. 5) starts with 1-O-galloyl-β-D-glucose (1-GG) as the first metabolite. This compound – more familiarly known as β-glucogallin – is formed via the esterification of gallic acid and activated UDP-glucose. In addition to being the first metabolite in the GG pathway, β-glucogallin also serves as the main acyl donor in the formation of other higher galloylated GGs; gallic acid as such is used only in the biosynthesis of β-glucogallin.
For instance, the biosynthesis of 1,6-di-O-galloyl-β-D-glucose (1,6-di-GG) needs two molecules of β-glucogallin, of which one serves as a galloyl donor and the other as an acceptor. Interestingly, also 1,6-di-GG has been shown to have the potential to donate its 1-galloyl moiety, especially for the formation of the next GG metabolite 1,2,6-tri-O-galloyl-β-D-glucose (1,2,6-tri-GG). Since both β-glucogallin and 1,6-di-GG require different acyltransferases in order to function as acyl donors in the galloylation processes, the choice of the acyl donor is based partly on the enzymes present in a plant. However, it has been suggested that the significance of β-glucogallin-independent reactions is negligible in comparison to the reactions involved in the main pathway, described in Fig. 5. Furthermore, Hagenah and & Gross (1993) found that the enzyme that catalysed the β-glucogallin-dependent galloylation of 1,2,6-tri-GG to 1,2,3,6-tetra-O-galloyl-β-D-glucose (1,2,3,6-tetra-GG), catalysed also the galloylation of 1,3,6-tri-O-galloylglucose to the same tetragalloylglucose, but with 12-fold efficiency. Because of these differences in reaction rates, it is possible that some intermediates of the main pathway are formed via an alternative route to that shown in Fig. 5, but these alternative intermediates cannot be found as major metabolites in plants due to their high turnover rates.
The formation of GTs from PGG has been evidenced by several enzymological studies (Hofmann and & Gross, 1990; Niemetz and & Gross, 1998, 1999, 2001). In all cases, the enzymes depended on the presence of 1-GG as the acyl donor. However, the high position-specificity found in the consecutive galloylations of simple GGs (1-galloyl > 6-galloyl > 2-galloyl > 3-galloyl 4-galloyl) is not as characteristic for the formation of GTs. Niemetz and & Gross (1999) found that the galloyltransferase most reactive in acylating PGG in the 4-position, acylated the 2-position instead, if the 4-position was already substituted with a digalloyl group. On the contrary, the galloyltransferase that preferentially galloylated PGG in the 3-position, catalysed further galloylation of 3-O-digalloyl-1,2,4,6-tetra-O-galloylglucose to afford the corresponding heptagalloylglucose being characterised by a 3-O-trigalloylgroup. The expected sequential synthesis of hexa,- hepta,- and octagalloylglucoses has been proven also by time-course experiments in vitro using enzyme preparations from leaves of Rhus typhina L. in the presence of PGG and β-glucogallin.